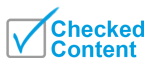
Ununtrium
Background Information
This wikipedia selection has been chosen by volunteers helping SOS Children from Wikipedia for this Wikipedia Selection for schools. SOS Children works in 45 African countries; can you help a child in Africa?
Ununtrium | |||||||||||||||||||||||||||||||||||||||||||
---|---|---|---|---|---|---|---|---|---|---|---|---|---|---|---|---|---|---|---|---|---|---|---|---|---|---|---|---|---|---|---|---|---|---|---|---|---|---|---|---|---|---|---|
113Uut
|
|||||||||||||||||||||||||||||||||||||||||||
|
|||||||||||||||||||||||||||||||||||||||||||
Appearance | |||||||||||||||||||||||||||||||||||||||||||
unknown | |||||||||||||||||||||||||||||||||||||||||||
General properties | |||||||||||||||||||||||||||||||||||||||||||
Name, symbol, number | ununtrium, Uut, 113 | ||||||||||||||||||||||||||||||||||||||||||
Pronunciation | / uː n ˈ uː n t r i ə m / oon-OON-tree-əm |
||||||||||||||||||||||||||||||||||||||||||
Metallic category | unknown presumably post-transition metals |
||||||||||||||||||||||||||||||||||||||||||
Group, period, block | 13, 7, p | ||||||||||||||||||||||||||||||||||||||||||
Standard atomic weight | [286] | ||||||||||||||||||||||||||||||||||||||||||
Electron configuration | [Rn] 5f14 6d10 7s2 7p1 (predicted) 2, 8, 18, 32, 32, 18, 3 (predicted) |
||||||||||||||||||||||||||||||||||||||||||
History | |||||||||||||||||||||||||||||||||||||||||||
Discovery | Joint Institute for Nuclear Research and Lawrence Livermore National Laboratory (2003) | ||||||||||||||||||||||||||||||||||||||||||
Physical properties | |||||||||||||||||||||||||||||||||||||||||||
Phase | solid (predicted) | ||||||||||||||||||||||||||||||||||||||||||
Density (near r.t.) | 18 (predicted) g·cm−3 | ||||||||||||||||||||||||||||||||||||||||||
Melting point | 700 K, 430 °C, 810 (predicted) °F | ||||||||||||||||||||||||||||||||||||||||||
Boiling point | 1400 K, 1100 °C, 2000 (predicted) °F | ||||||||||||||||||||||||||||||||||||||||||
Heat of vaporization | 130 (predicted) kJ·mol−1 | ||||||||||||||||||||||||||||||||||||||||||
Atomic properties | |||||||||||||||||||||||||||||||||||||||||||
Oxidation states | 1, 2, 3, 5 (prediction) | ||||||||||||||||||||||||||||||||||||||||||
Ionization energies | 1st: 704.9 (prediction) kJ·mol−1 | ||||||||||||||||||||||||||||||||||||||||||
Atomic radius | 170 (prediction) pm | ||||||||||||||||||||||||||||||||||||||||||
Covalent radius | 136 (prediction) pm | ||||||||||||||||||||||||||||||||||||||||||
Miscellanea | |||||||||||||||||||||||||||||||||||||||||||
CAS registry number | 54084-70-7 | ||||||||||||||||||||||||||||||||||||||||||
Most stable isotopes | |||||||||||||||||||||||||||||||||||||||||||
Main article: Isotopes of ununtrium | |||||||||||||||||||||||||||||||||||||||||||
|
|||||||||||||||||||||||||||||||||||||||||||
Ununtrium is the temporary name of a chemical element with the temporary symbol Uut and atomic number 113. It is an extremely radioactive synthetic element (an element that can be created in a laboratory but is not found in nature); the most stable known isotope, ununtrium-286, has a half-life of 20 seconds. Ununtrium was first created in 2003 by the Joint Institute for Nuclear Research in Dubna, Russia.
In the periodic table, it is a p-block transactinide element. It is a member of the 7th period and is placed in the boron group, although no chemical experiments have been carried out to confirm that it behaves as the heavier homologue to thallium in the boron group. Ununtrium is calculated to have some similar properties to its lighter homologues, boron, aluminium, gallium, indium, and thallium, although it should also show several major differences from them. Unlike all the other p-block elements, it is even predicted to show some transition metal character.
History
Dubna–Livermore collaboration
The first report of ununtrium was in August 2003 when it was identified as an alpha decay product of element 115, ununpentium. These results were published on February 1, 2004, by a team composed of Russian scientists at Dubna ( Joint Institute for Nuclear Research), and American scientists at the Lawrence Livermore National Laboratory:
- 243
95Am + 48
20Ca → 288
115Uup + 3 1
0n → 284
113Uut + α - 243
95Am + 48
20Ca → 287
115Uup + 4 1
0n → 283
113Uut + α
The Dubna–Livermore collaboration has strengthened their claim for the discovery of ununtrium by conducting chemical experiments on the final decay product 268Db. In experiments in June 2004 and December 2005, the dubnium isotope was successfully identified by extracting the final decay products, measuring spontaneous fission (SF) activities and using chemical identification techniques to confirm that they behave like a group 5 element (as dubnium is known to be in group 5 of the periodic table). Both the half-life and decay mode were confirmed for the proposed 268Db which lends support to the assignment of the parent and daughter nuclei to ununpentium and ununtrium respectively. Further experiments at Dubna in 2005 have fully confirmed the decay data for ununpentium and ununtrium, but in 2011, the IUPAC/IUPAP Joint Working Party (JWP) did not recognize the two elements as having been discovered because current theory could not distinguish between group 4 and group 5 elements by their properties with sufficient confidence, and the identification of the daughter dubnium isotope was the most important factor in confirming the discovery of ununpentium and ununtrium.
RIKEN
On July 23, 2004, a team of Japanese scientists at RIKEN bombarded a target of bismuth-209 with accelerated nuclei of zinc-70 and detected a single atom of the isotope ununtrium-278. They published their results on September 28, 2004:
- 209
83Bi + 70
30Zn → 278
113Uut + 1
0n
Previously, in 2000, a team led by P. A. Wilk identified the decay product 266Bh as decaying with identical properties to what the Japanese team had observed, thus lending support for their claim. However, they also observed the daughter of 266Bh, 262Db, undergo alpha decay instead of spontaneous fission (which the Japanese team observed).
The RIKEN team produced a further atom on April 2, 2005, although the decay data were slightly different from the first chain, perhaps due to either the formation of a metastable state or an alpha particle escaping from the detector before depositing its full energy. Due to these inconsistencies in the decay data, the small number of ununtrium atoms produced, and the lack of unambiguous anchors to known isotopes, the JWP did not accept this as a conclusive discovery of ununtrium in 2011.
Most recently, production and identification of another 278Uut nucleus occurred at RIKEN on August 12, 2012. In this case, a series of six alpha decays was observed:
- 278
113Uut → 274
111Rg + α → 270
109Mt + α → 266
107Bh + α → 262
105Db + α → 258
103Lr + α → 254
101Md + α
This decay chain differed from the previous observations at RIKEN mainly in the decay mode of dubnium, which was previously observed to undergo spontaneous fission, but in this case instead alpha decayed. Because the alpha decay of dubnium-262 to lawrencium-258 is well known, this provides unambiguous proof that element 113 is the origin of the chain. The scientists on this team calculated the probability of accidental coincidence to be 10−28, or totally negligible.
Naming
Ununtrium is the lightest element that has not yet received an official name. Using Mendeleev's nomenclature for unnamed and undiscovered elements, ununtrium should be known as eka-thallium or dvi-indium. In 1979 IUPAC published recommendations according to which the element was to be called ununtrium (with the corresponding symbol of Uut), a systematic element name as a placeholder, until the discovery of the element is confirmed and a name is decided on. The recommendations are largely ignored among scientists, who call it "element 113", with the symbol of (113) or even simply 113.
Claims to the discovery of ununtrium have been put forward by both the Dubna and RIKEN teams. The IUPAC/IUPAP Joint Working Party (JWP) will decide to whom the right to suggest a name will be given. In 2011, the IUPAC evaluated the 2004 RIKEN experiments and 2004 and 2007 Dubna experiments, and concluded that they did not meet the criteria for discovery.
The following names have been suggested by the above-mentioned teams claiming discovery:
Group | Proposed name | Derivation |
---|---|---|
RIKEN | Japonium | Japan: country of group claimants |
Rikenium | RIKEN: institute of group claimants | |
Nishinanium | Yoshio Nishina, Japanese physicist |
Nucleosynthesis
Super-heavy elements such as ununtrium are produced by bombarding lighter elements in particle accelerators that induce fusion reactions. Whereas most of the isotopes of ununtrium can be synthesized directly this way, some heavier ones have only been observed as decay products of elements with higher atomic numbers.
Depending on the energies involved, the former are separated into "hot" and "cold". In hot fusion reactions, very light, high-energy projectiles are accelerated toward very heavy targets (actinides), giving rise to compound nuclei at high excitation energy (~40–50 MeV) that may either fission or evaporate several (3 to 5) neutrons. In cold fusion reactions, the produced fused nuclei have a relatively low excitation energy (~10–20 MeV), which decreases the probability that these products will undergo fission reactions. As the fused nuclei cool to the ground state, they require emission of only one or two neutrons, and thus, allows for the generation of more neutron-rich products. The latter is a distinct concept from that of where nuclear fusion claimed to be achieved at room temperature conditions (see cold fusion).
Cold fusion
Before the successful synthesis of ununtrium by the RIKEN team, scientists at the Institute for Heavy Ion Research (Gesellschaft für Schwerionenforschung) in Darmstadt, Germany also tried to synthesize ununtrium by bombarding bismuth-209 with zinc-70 in 1998. No ununtrium atoms were identified in two separate runs of the reaction. They repeated the experiment in 2003 again without success. In late 2003, the emerging team at RIKEN using their efficient apparatus GARIS attempted the reaction and reached a limit of 140 fb. In December 2003 – August 2004, they resorted to "brute force" and carried out the reaction for a period of eight months. They were able to detect a single atom of 278Uut. They repeated the reaction in several runs in 2005 and were able to synthesize a second atom.
Hot fusion
In June 2006, the Dubna-Livermore team synthesised ununtrium directly by bombarding a neptunium-237 target with accelerated calcium-48 nuclei:
- 237
93Np + 48
20Ca → 282
113Uut + 1
0n
Two atoms of 282Uut were detected.
As decay product
Evaporation residue | Observed ununtrium isotope |
---|---|
294Uus, 290Uup | 286Uut |
293Uus, 289Uup | 285Uut |
288Uup | 284Uut |
287Uup | 283Uut |
Ununtrium has been observed as decay products of ununpentium. Ununpentium currently has four known isotopes; all of them undergo alpha decays to become ununtrium nuclei, with mass numbers between 283 and 286. Parent ununpentium nuclei can be themselves decay products of ununseptium. To date, no other elements have been known to decay to ununtrium. For example, in January 2010, the Dubna team ( JINR) identified ununtrium-286 as a product in the decay of ununseptium via an alpha decay sequence:
- 294
117Uus → 290
115Uup + 4
2He - 290
115Uup → 286
113Uut + 4
2He
Isotopes
Isotope |
Half-life |
Decay mode |
Discovery year |
Reaction |
---|---|---|---|---|
278Uut | 0.24 ms | α | 2004 | 209Bi(70Zn,n) |
282Uut | 70 ms | α | 2006 | 237Np(48Ca,3n) |
283Uut | 0.10 s | α | 2003 | 287Uup(—,α) |
284Uut | 0.48 s | α | 2003 | 288Uup(—,α) |
285Uut | 5.5 s | α | 2009 | 293Uus(—,2α) |
286Uut | 20 s | α | 2009 | 294Uus(—,2α) |
287Uut | 20? min | α, SF ? | unknown | — |
Ununtrium has no stable or naturally-occurring isotopes. Several radioactive isotopes have been synthesized in the laboratory, either by fusing two atoms or by observing the decay of heavier elements. Six different isotopes of ununtrium have been reported with atomic masses 278 and 282–286; they all decay through alpha decay.
Stability and half-lives
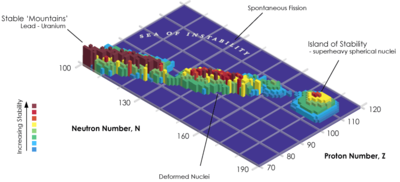
All ununtrium isotopes are extremely unstable and radioactive; however, the heavier ununtrium isotopes are more stable than the lighter. The most stable known ununtrium isotope, 286Uut, is also the heaviest known ununtrium isotope; it has a half-life of 20 seconds. The isotope 285Uut has been reported to also have a half-life of over a second. The isotopes 284Uut and 283Uut have half-lives of 0.48 and 0.10 seconds respectively. The remaining two isotopes have half-lives between 0.1 and 100 milliseconds: 282Uut has a half-life of 70 milliseconds, and 278Uut, the lightest known ununtrium isotope, is also the shortest-lived known ununtrium isotope, with a half-life of just 0.24 milliseconds. It is predicted that even heavier undiscovered ununtrium isotopes could be much more stable: for example, 287Uut is predicted to have a half-life of around 20 minutes, close to two orders of magnitude more than that of 286Uut.
Theoretical estimates of alpha decay half-lives of isotopes of ununtrium are in good agreement with the experimental data. The undiscovered isotope 293Uut has been predicted to be the most stable towards beta decay; however, no known ununtrium isotope has been observed to undergo beta decay.
The stability of nuclei decreases greatly with the increase in atomic number after plutonium, the heaviest primordial element, so that all isotopes with an atomic number above 101 decay radioactively with a half-life under a day, with the exception of dubnium-268. No elements with atomic numbers above 82 (after lead) have stable isotopes. Nevertheless, because of reasons not very well understood yet, there is a slight increased nuclear stability around atomic numbers 110–114, which leads to the appearance of what is known in nuclear physics as the " island of stability". This concept, proposed by University of California professor Glenn Seaborg, explains why superheavy elements last longer than predicted.
Predicted properties
Ununtrium is the first member of the 7p series of elements and the heaviest boron group element on the periodic table, below boron, aluminium, gallium, indium, and thallium. It is predicted to show many differences from its lighter homologues: a largely contributing effect is the spin–orbit (SO) interaction. It is especially strong for the superheavy elements, because their electrons move much faster than in lighter atoms, at velocities comparable to the speed of light, which is where the differences arise from. In relation to ununtrium atoms, it lowers the 7s and the 7p electron energy levels (stabilizing the corresponding electrons), but two of the 7p electron energy levels are stabilized more than the other four. The stabilization of the 7s electrons is called the inert pair effect, and the effect "tearing" the 7p subshell into the more stabilized and the less stabilized parts is called the subshell splitting. Computation chemists see the split as a change of the second ( azimuthal) quantum number l from 1 to 1/2 and 3/2 for the more stabilized and less stabilized parts of the 7p subshell, respectively. For many theoretical purposes, the valence electron configuration may be represented to reflect the 7p subshell split as 7s27p1/21. These effects stabilize lower oxidation states: the first ionization energy of ununtrium is expected to be 7.306 eV, the highest among the boron group elements. Hence, the most stable oxidation state of ununtrium is predicted to be the +1 state. Differences for other electron levels also exist. For example, the 6d electron levels (also split in halves, with four being 6d3/2 and six being 6d5/2) are both raised, so that they are close in energy to the 7s ones. Thus, the 6d electron levels, being destabilized, should be able to participate in chemical reactions in the earlier 7p elements (until around ununpentium), thus making them behave in some ways like transition metals and allow higher oxidation states. Ununtrium should hence also be able to show stable +2, +3 and +5 oxidation states. However, the +3 state should still be less stable than the +1 state, following periodic trends. Ununtrium should be the most electronegative among all the boron group elements: for example, in the compound Uut Uus, the negative charge is expected to be on the ununtrium atom rather than the ununseptium atom, the opposite of what would be expected from simple periodicity. The electron affinity of ununtrium is calculated to be around 0.68 eV; in comparison, that of thallium is 0.4 eV. The high electron affinity and electronegativity of ununtrium are due to it being only one electron short of the closed-shell valence electron configuration of flerovium (7s27p1/22).
The simplest possible ununtrium compound is the monohydride, UutH. The bonding is provided by the 7p1/2 electron of ununtrium and the 1s electron of hydrogen. However, the SO interaction causes the binding energy of ununtrium monohydride to be reduced by about 1 eV and the ununtrium–hydrogen bond length to decrease as the bonding 7p1/2 orbital is relativistically contracted. The analogous monofluoride (UutF) should also exist. Ununtrium should also be able to form the trihydride (UutH3), trifluoride (UutF3), and trichloride (UutCl3), with ununtrium in the +3 oxidation state. Because the 6d electrons are involved in bonding instead of the 7s ones, these molecules are predicted to be T-shaped and not trigonal planar. Although the polyfluoride anion UutF−
6 should be stable, the corresponding neutral fluoride UutF5 should be unstable, spontaneously decomposing into the trifluoride and elemental fluorine. Ununtrium(I) is predicted to be more similar to silver(I) than thallium(I).
Ununtrium is expected to be much denser than thallium, having a predicted density of about 18 g/cm3, due to the relativistic stabilization and contraction of its 7s and 7p1/2 orbitals. This is because calculations estimate it to have an atomic radius of about 170 pm, the same as that of thallium, even though periodic trends would predict it to have an atomic radius larger than that of thallium due to it being one period further down in the periodic table. The melting and boiling points of ununtrium are not definitely known, but have been calculated to be 430 °C and 1100 °C respectively, exceeding the values for gallium, indium, and thallium, following periodic trends.