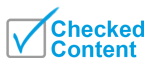
Fluorine
Did you know...
SOS Children made this Wikipedia selection alongside other schools resources. A good way to help other children is by sponsoring a child
Fluorine | ||||||||||||||||||||||||||||
---|---|---|---|---|---|---|---|---|---|---|---|---|---|---|---|---|---|---|---|---|---|---|---|---|---|---|---|---|
9F
|
||||||||||||||||||||||||||||
|
||||||||||||||||||||||||||||
Appearance | ||||||||||||||||||||||||||||
gas: very pale yellow liquid: bright yellow solid: transparent (beta), opaque (alpha) ![]() Liquid fluorine at cryogenic temperatures |
||||||||||||||||||||||||||||
General properties | ||||||||||||||||||||||||||||
Name, symbol, number | fluorine, F, 9 | |||||||||||||||||||||||||||
Pronunciation | / ˈ f l ʊər iː n /, / ˈ f l ʊər ɪ n /, / ˈ f l ɔər iː n / | |||||||||||||||||||||||||||
Element category | halogen | |||||||||||||||||||||||||||
Group, period, block | 17 (halogens), 2, p | |||||||||||||||||||||||||||
Standard atomic weight | 18.9984032(5) | |||||||||||||||||||||||||||
Electron configuration | [He] 2s2 2p5 2, 7 |
|||||||||||||||||||||||||||
History | ||||||||||||||||||||||||||||
Discovery | André-Marie Ampère (1810) | |||||||||||||||||||||||||||
First isolation | Henri Moissan (June 26, 1886) | |||||||||||||||||||||||||||
Named by | Humphry Davy | |||||||||||||||||||||||||||
Physical properties | ||||||||||||||||||||||||||||
Phase | gas | |||||||||||||||||||||||||||
Density | (0 °C, 101.325 kPa) 1.696 g/L |
|||||||||||||||||||||||||||
Liquid density at b.p. | 1.505 g·cm−3 | |||||||||||||||||||||||||||
Melting point | 53.53 K, −219.62 °C, −363.32 °F | |||||||||||||||||||||||||||
Boiling point | 85.03 K, −188.12 °C, −306.62 °F | |||||||||||||||||||||||||||
Critical point | 144.4 K, 5.215 MPa | |||||||||||||||||||||||||||
Heat of vaporization | 6.51 kJ·mol−1 | |||||||||||||||||||||||||||
Molar heat capacity | (Cp) (21.1 °C) 825 J·mol−1·K−1 (Cv) (21.1 °C) 610 J·mol−1·K−1 |
|||||||||||||||||||||||||||
Vapor pressure | ||||||||||||||||||||||||||||
|
||||||||||||||||||||||||||||
Atomic properties | ||||||||||||||||||||||||||||
Oxidation states | −1 (oxidizes oxygen) |
|||||||||||||||||||||||||||
Electronegativity | 3.98 (Pauling scale) | |||||||||||||||||||||||||||
Ionization energies ( more) |
1st: 1,681 kJ·mol−1 | |||||||||||||||||||||||||||
2nd: 3,374 kJ·mol−1 | ||||||||||||||||||||||||||||
3rd: 6,147 kJ·mol−1 | ||||||||||||||||||||||||||||
Covalent radius | 64 pm | |||||||||||||||||||||||||||
Van der Waals radius | 135 pm | |||||||||||||||||||||||||||
Miscellanea | ||||||||||||||||||||||||||||
Crystal structure | cubic
|
|||||||||||||||||||||||||||
Magnetic ordering | diamagnetic | |||||||||||||||||||||||||||
Thermal conductivity | 0.02591 W·m−1·K−1 | |||||||||||||||||||||||||||
CAS registry number | 7782-41-4 | |||||||||||||||||||||||||||
Most stable isotopes | ||||||||||||||||||||||||||||
Main article: Isotopes of fluorine | ||||||||||||||||||||||||||||
|
||||||||||||||||||||||||||||
Fluorine (symbol F) is the chemical element with atomic number 9. It is the lightest halogen. At standard pressure and temperature, fluorine is a pale yellow gas composed of diatomic molecules, F2. Fluorine is the most electronegative element and is extremely reactive, requiring great care in handling. It has a single stable isotope, fluorine-19.
In stars, fluorine is rare compared to other light elements. In Earth's crust, fluorine is the thirteenth most abundant element. Fluorine's most important mineral, fluorite, was first formally described in 1530, in the context of smelting. The mineral's name derives from the Latin verb fluo, which means "flow", because fluorite was added to metal ores to lower their melting points. Suggested as a chemical element in 1811, fluorine was named after the source mineral, but resisted many attempts to isolate the element. In 1886, French chemist Henri Moissan succeeded. His method of electrolysis remains the industrial production method for fluorine gas. The main use of elemental fluorine, uranium enrichment, was developed during the Manhattan Project.
Because of the difficulty in making elemental fluorine, most fluorine used in commerce is never converted to free fluorine. Instead, hydrofluoric acid is the key intermediate for the $16 billion per year global fluorochemical industry. The fluorides of low charged metals are ionic compounds (salts); those of high charged metals are volatile molecular compounds. The largest uses of inorganic fluorides are steel making and aluminium refining.
Organic fluorine compounds tend to have high chemical and thermal stability. The largest commercial use is in refrigerant gases (the many types of "Freons"). Although traditional chlorofluorocarbons are widely banned, the replacement gases still contain fluorine. Polytetrafluoroethylene (Teflon) is the most important fluoropolymer and is used in electrical insulation, chemical-resistant parts, stadium roofs, and cookware. A growing fraction of modern pharmaceuticals contain fluorine; Lipitor and Prozac are prominent examples. While a few plants and bacteria synthesize organofluorine poisons, fluorine has no metabolic role in mammals. The fluoride ion, when directly applied to teeth, reduces decay and for this reason is used in toothpaste and municipal water fluoridation.
Characteristics
Physical properties
Fluorine forms diatomic molecules that are gaseous at room temperature. The density is about 1.3 times that of air. Though sometimes cited as yellow-green, fluorine gas is actually a very pale yellow. Its color can only be observed in concentrated fluorine gas when looking down the axis of long tubes. It appears transparent when observed from the side in normal glass tubes or if allowed to escape into the atmosphere. The element has a "pungent" characteristic odour that is noticeable in concentrations as low as 20 ppb.
Fluorine condenses to a bright yellow liquid at −188 °C (−307 °F), close to the condensation temperatures of oxygen and nitrogen. Fluorine solidifies at −220 °C (−363 °F) into a cubic structure, called beta-fluorine. This phase is transparent and soft, with significant disorder of the molecules. At −228 °C (−378 °F) fluorine undergoes a solid–solid phase transition into a monoclinic structure called alpha-fluorine. This phase is opaque and hard with close-packed layers of molecules. The solid state phase change requires more energy than the melting point transition and can be violent, shattering samples and blowing out sample holder windows. In general, fluorine's solid state is more similar to oxygen's than to the other halogens'.
![]() |
![]() |
Low-temperature fluorine phases | Alpha-fluorine crystal structure |
Atomic structure
A fluorine atom has nine protons and nine electrons, one fewer than neon, arranged in the electronic configuration [He]2s22p5. Fluorine's outer electrons are relatively separate from each other, and thus they do not shield each other from the nucleus. Therefore, they experience a high effective nuclear charge. Fluorine has a relatively small covalent radius, slightly less than 60 picometers, which is less than its neighbors, oxygen and neon.
Fluorine is reluctant to ionize and instead has an attraction for one more electron to achieve the extremely stable neon-like arrangement. Fluorine's first ionization energy (energy required to remove an electron to form F+) is 1,681 kilojoules per mole, which is higher than for any other element except neon and helium. Fluorine's electron affinity (energy released by adding an electron to form F–) is 328 kilojoules per mole, which is higher than that of any other element except chlorine.
Molecular structure
Although an individual fluorine atom has one unpaired electron, in molecular fluorine all the electrons are paired. Because of this, elemental fluorine is diamagnetic (slightly repelled by magnets). In contrast, the molecules of neighbour element oxygen, with two unpaired electrons per molecule, are paramagnetic (attracted to magnets). The measured value of fluorine's magnetic susceptibility is −9.6×10−6 (cgs), which is close to theoretical predictions. The experimental result was not accomplished until 1999 because of the difficulties in handling fluorine gas as well as the need to specially purify the fluorine of any trace of paramagnetic oxygen.
The fluorine–fluorine bond of the difluorine molecule is relatively weak when compared to the bond of the other halogens. The bond energy is significantly weaker than those of dichlorine or dibromine molecules and similar to the easily cleaved oxygen–oxygen bonds of peroxides or nitrogen–nitrogen bonds of hydrazines. The covalent radius of fluorine in difluorine molecules, about 71 picometers, is significantly larger than that in other compounds because of the weak bonding between fluorine atoms. This happens because the electron and internuclear repulsions are relatively great, and the bonding orbital overlap is decreased due to the small size of the atoms.
Fluorine's 2p electrons are purely 2p, and the difluorine molecule has exactly one bond (a bond order of exactly 1). In contrast, the heavier halogens' p electrons levels partly hybridize with those of d electrons. This makes them behave partly like d electrons, and this partial d character allows to show partial bonding between d electrons. Therefore, the heavier halogens have a bond order effectively greater than 1; for example, chlorine has a bond order of 1.12. Fluorine's electrons cannot show this d character because there are no d electrons close in energy to fluorine's 2p.
X | X2 | HX | BX3 | AlX3 | CX4 |
---|---|---|---|---|---|
F | 159 | 574 | 645 | 582 | 456 |
Cl | 243 | 428 | 444 | 427 | 327 |
Br | 193 | 363 | 368 | 360 | 272 |
I | 151 | 294 | 272 | 285 | 239 |
Chemical reactivity
Fluorine's chemistry is dominated by its tendency to gain an electron. It is the most electronegative element and a strong oxidant. The removal of an electron from a fluorine atom requires so much energy that no known oxidant can oxidize fluorine to any positive oxidation state.
Reactions with fluorine are often sudden or explosive. Many generally non-reactive substances such as powdered steel, glass fragments and asbestos fibers are readily consumed by cold fluorine gas. Wood and even water burn with flames when subjected to a jet of fluorine, without the need for a spark.
Fluorine reacting with caesium, video by the Royal Institution. (Both videos filmed at a fluorine laboratory of the University of Leicester.) |
Fluorine forms compounds, fluorides, with all elements except neon and helium. All of the elements up to einsteinium, element 99, have been checked except for astatine and francium. Fluorine is also known to form compounds with rutherfordium, element 104, and seaborgium, element 106. Several heavy radioactive elements have not been fluoridated because of their extreme rarity, but such reactions are theoretically possible.
All metals react with fluorine, but conditions vary with the metal. Often, the metal must be powdered because many metals passivate (form protective layers of the metal fluoride that resist further fluoridation). The alkali metals react with fluorine with a bang (small explosion), while the alkaline earth metals react at room temperature as well but not as aggressively. The noble metals ruthenium, rhodium, palladium, platinum, and gold react least readily, requiring pure fluorine gas at 300–450 °C (575–850 °F).
Fluorine reacts explosively with hydrogen in a manner similar to that of alkali metals. The halogens react readily with fluorine gas as does the heavy noble gas radon. The lighter noble gases xenon and krypton can be made to react with fluorine under special conditions and argon will combine with hydrogen fluoride. Nitrogen, with its very stable triple bonds, requires electric discharge and high temperatures to combine directly with fluorine.
Isotopes
Fluorine occurs naturally on Earth exclusively in the form of its only stable isotope, fluorine-19, which makes the element monoisotopic and mononuclidic. Seventeen radioisotopes have been synthesized: mass numbers 14–18 and 20–31. Fluorine-18 is the most stable radioisotope of fluorine, with a half-life of 109.77 minutes. It is also the lightest unstable nuclide with equal odd numbers of protons and neutrons.
The lightest fluorine isotopes, those with mass numbers of 14–16, decay via electron capture. 17F and 18F undergo beta plus decay (positron emission). All isotopes heavier than the stable fluorine-19 decay by beta minus mode (electron emission). Some of them also decay by neutron emission.
Only one nuclear isomer (long-lived excited nuclear state), fluorine-18m, has been characterized. Its half-life before gamma ray emission is 160 nanoseconds. This is less than the decay half-life of any of the fluorine radioisotope nuclear ground states except numbers 14–16, 28, and 31.
Origin and occurrence
In the universe
Atomic number |
Element | Relative amount |
---|---|---|
6 | Carbon | 4,800 |
7 | Nitrogen | 1,500 |
8 | Oxygen | 8,800 |
9 | Fluorine | 1 |
10 | Neon | 1,400 |
11 | Sodium | 24 |
12 | Magnesium | 430 |
From the perspective of cosmology, fluorine is relatively rare with 400 ppb in the universe. Within stars, any fluorine that is created is rapidly eliminated through nuclear fusion: either with hydrogen to form oxygen and helium, or with helium to make neon and hydrogen. The presence of fluorine at all—outside of temporary existence in stars—is somewhat of a mystery because of the need to escape these fluorine-destroying reactions.
Three theoretical solutions to the mystery exist. In type II supernovae, atoms of neon are hit by neutrinos during the explosion and converted to fluorine. In Wolf-Rayet stars (blue stars over 40 times heavier than the Sun), a strong solar wind blows the fluorine out of the star before hydrogen or helium can destroy it. In asymptotic giant branch (a type of red giant) stars, fusion reactions occur in pulses and convection lifts fluorine out of the inner star. Only the red giant hypothesis has supporting evidence from observations.
In space, fluorine commonly combines with hydrogen to form hydrogen fluoride. (This compound has been suggested as a proxy to enable tracking reservoirs of hydrogen in the universe.) In addition to HF, monatomic fluorine has been observed in the interstellar medium. Fluorine cations have been seen in planetary nebulae and in stars, including our Sun.
On Earth
Fluorine is the thirteenth most common element in Earth's crust, comprising between 600 and 700 ppm of the crust by mass. Because of its reactivity, it is essentially only found in compounds. Three minerals exist that are industrially relevant sources: fluorite, fluorapatite, and cryolite.
- Fluorite (CaF2), also called fluorspar or Blue John, is the main source of commercial fluorine. Fluorite is a colorful mineral associated with hydrothermal deposits. It is common and found worldwide. China supplies more than half of the world's demand; Mexico is the second-largest producer. The United States produced most of the world's fluorite in the early 20th century, but the last mine, in Illinois, shut down in 1995.
- Fluorapatite (Ca5(PO4)3F) is mined along with other apatites for its phosphate content and is used mostly for production of fertilizers. Most of the Earth's fluorine is bound in this mineral, but because the percentage within the mineral is low (3.5%), the fluorine is discarded as waste. Only in the United States is there significant recovery. There the hexafluorosilicates produced as byproducts are used to supply municipal water fluoridation.
- Cryolite (Na3AlF6) is the least abundant of the three, but is a concentrated source of fluorine. It was formerly used directly in aluminium production. However, the main commercial mine, on the west coast of Greenland, closed in 1987.
Major fluorine-containing minerals | ||
![]() |
![]() |
![]() |
Fluorite | Fluorapatite | Cryolite |
Several other minerals, such as the gemstone topaz, contain fluoride. Fluoride is not significant in seawater or brines, unlike the other halides, because the alkaline earth fluorides precipitate out of water.
Organofluorines have been observed in volcanic eruptions and in geothermal springs. Their ultimate origin is said to vary from physical formation under geological conditions to initial biological production and deposition in sediments. However, the provenance is still being studied, as is the natural organofluorine distribution. They are not found in large quantites (compare also the number of known natural organofluorines, 30, to that of organochlorines, 2150), so they are not commercially important source of fluorine.
The possibility of small amounts of gaseous fluorine within crystals has been debated for many years. One form of fluorite, antozonite, has a smell suggestive of fluorine when crushed. The mineral also has a dark black colour, perhaps from free calcium (not bonded to fluoride). In 2012, a study reported detection of trace quantities (0.04% by weight) of diatomic fluorine in antozonite. It was suggested that radiation from small amounts of uranium within the crystals had caused the free fluorine defects.
History
The word "fluorine" derives from the Latin stem of the main source mineral, fluorite, which was first mentioned in 1529 by Georgius Agricola, who described it as a flux—an additive that helps melt ores and slags during smelting. Fluorite stones were called schone flusse in the German of the time. Agricola, writing in Latin but describing 16th century industry, invented several hundred new Latin terms. For the schone flusse stones, he used the Latin noun fluores, "fluxes", because they made metal ores flow when in a fire. After Agricola, the name for the mineral evolved to fluorspar (still commonly used) and then to fluorite.
Some sources claim that the first production of hydrofluoric acid was by Heinrich Schwanhard, a German glass cutter, in 1670. A peer-reviewed study of Schwanhard's writings, though, showed no specific mention of fluorite and only discussion of an extremely strong acid. It was hypothesized that this was probably nitric acid or aqua regia, both capable of etching soft glass. Andreas Sigismund Marggraf made the first recorded preparation of hydrofluoric acid in 1764 when he heated fluorite with sulfuric acid in glass, which was greatly corroded by the product. In 1771, Swedish chemist Carl Wilhelm Scheele repeated this reaction. Scheele recognized the product of the reaction as an acid, which he called "fluss-spats-syran" (fluor-spar-acid); in English, it was known as "fluoric acid". In 1810, French physicist André-Marie Ampère suggested that the acid was a compound of hydrogen with an unknown element, analogous to chlorine. Fluorite was then shown to be mostly composed of calcium fluoride.
Sir Humphry Davy originally suggested the name fluorine, taking the root from the name of "fluoric acid" and the -ine suffix, similarly to other halogens. This name, with modifications, came to most European languages. (Greek, Russian, and several other languages use the name ftor or derivatives, which was suggested by Ampère and comes from the Greek φθόριος (phthorios), meaning "destructive".) The New Latin name (fluorum) gave the element its current symbol, F, although the symbol Fl is seen in early papers. The symbol Fl is now used for the super-heavy element flerovium.
Owing to its extreme reactivity, elemental fluorine was not isolated until many years after the characterization of fluorite. Progress in isolating elemental fluorine was slow because its electrolytical preparation was hard to perform and because the gas reacted with most materials. The generation of elemental fluorine proved to be exceptionally dangerous, killing or blinding several early experimenters. Jean Dussaud referred to these men as "fluorine martyrs", a term still used.
Edmond Frémy thought that passing electric current through pure hydrofluoric acid might work. Previously, hydrogen fluoride was only available in a water solution. Frémy therefore devised a method for producing dry hydrogen fluoride by acidifying potassium bifluoride (KHF2). Unfortunately, pure hydrogen fluoride did not pass an electric current.
French chemist Henri Moissan, formerly one of Frémy's students, continued the search. After trying many different approaches, he built on Frémy's earlier attempt by combining potassium bifluoride and hydrogen fluoride. The resultant solution conducted electricity. Moissan also constructed especially corrosion-resistant equipment: containers crafted from a mixture of platinum and iridium (more chemically resistant than pure platinum) with fluorite stoppers. After 74 years of effort by many chemists, on 26 June 1886, Moissan reported the isolation of elemental fluorine. Moissan's report to the French Academy of making fluorine showed appreciation for the feat:
One can indeed make various hypotheses on the nature of the liberated gas; the simplest would be that we are in the presence of fluorine
Moissan later devised a less expensive apparatus for making fluorine: copper equipment coated with copper fluoride. In 1906, two months before his death, Moissan received the Nobel Prize in chemistry for his fluorine isolation as well as the invention of the electric arc furnace.
During the 1930s and 1940s, the DuPont company commercialized organofluorine compounds at large scales. Following trials of chlorofluorcarbons as refrigerants by researchers at General Motors, DuPont developed large-scale production of Freon-12. DuPont and GM formed a joint venture in 1930 to market the new product; in 1949 DuPont took over the business. Freon proved to be a marketplace hit, rapidly replacing earlier, more toxic, refrigerants and growing the overall market for kitchen refrigerators.
In 1938, polytetrafluoroethylene (Teflon) was discovered by accident by a recently-hired DuPont PhD, Roy J. Plunkett. While working with a cylinder of tetrafluoroethylene, he was unable to release the gas, although the weight had not changed. Scraping down the container, he found white flakes of a polymer new to the world. Tests showed the substance was resistant to corrosion from most substances and had better high temperature stability than any other plastic. By early 1941, a crash program was making commercial quantities.
Large-scale productions of elemental fluorine began during World War II. Germany used high-temperature electrolysis to produce tons of chlorine trifluoride, a compound planned to be used as an incendiary. The Manhattan project in the United States produced even more fluorine for use in uranium separation. Gaseous uranium hexafluoride was used to separate uranium-235, an important nuclear explosive, from the heavier uranium-238 in centrifuges and diffusion plants. Because uranium hexafluoride releases small quantities of corrosive fluorine, the separation plants were built with special materials. All pipes were coated with nickel; joints and flexible parts were fabricated from Teflon.
In 1958, a DuPont research manager in the Teflon business, Bill Gore, left the company because of its unwillingess to develop Teflon as wire-coating insulation. Gore's son Robert found a method for solving the wire-coating problem and the company W. L. Gore and Associates was born. In 1969, Robert Gore developed an expanded PTFE membrane which led to the large Gore-tex business in breathable rainwear. The company developed many other uses of PTFE.
In the 1970s and 1980s, concerns developed over the role chlorofluorocarbons play in damaging the ozone layer. By 1996, almost all nations had banned chlorofluorocarbon refrigerants and commercial production ceased. Fluorine continued to play a role in refrigeration though: hydrochlorofluorocarbons (HCFCs) and hydrofluorocarbons (HFCs) were developed as replacement refrigerants.
Industry and applications
The global market for fluorochemicals was about $16 billion per year as of 2006. Historically, the industry has grown a few percent per year and is predicted to do so in the future. Although fluorochemical demand contracted during the 2008–2009 global recession, the industry was predicted to reach 2.6 million tons per year by 2015.
The largest market is the United States. Western Europe is the second largest. Asia Pacific is the fastest growing region of production. China in particular has experienced significant growth as a fluorochemical market and is becoming a producer of them as well.
Fluorite mining (the main source of fluorine) was estimated in 2003 to be a $550 million industry, extracting 4.5 million tons per year. Most ores must be processed to concentrate the fluorite from other minerals by various methods of flotation separation. However, only about 1% of mined fluorite is ever converted to elemental fluorine.
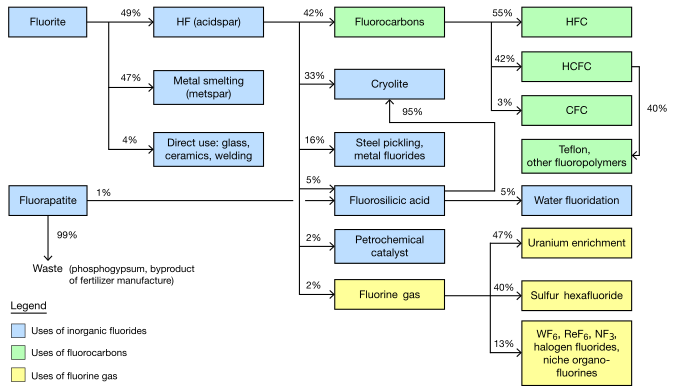

Inorganic fluorides
Mined fluorite is separated into two main grades, with about equal production of each. Acidspar is at least 97% CaF2; metspar is much lower purity, 60–85%. (A small amount of the intermediate, ceramic, grade is also made.)
Metspar is used almost exclusively for iron smelting. Acidspar is primarily converted to hydrofluoric acid (by reaction with sulfuric acid). The resultant HF is mostly used to produce organofluorides and synthetic cryolite.
About 3 kg (6.5 lb) of metspar grade fluorite, added directly to the batch, are used for every tonne of steel made. The fluoride ions from CaF2 lower the melt's temperature and viscosity (make the liquid runnier). The calcium content has a tangential benefit in removing sulfur and phosphorus, but other additives such as lime are still needed. Metspar is similarly used in cast iron production and for other iron-containing alloys.
Fluorite of the acidspar grade is used directly as an additive to ceramics and enamels, glass fibers and clouded glass, and cement, as well as in the outer coating of welding rods. Acidspar is primarily used for making hydrofluoric acid, which is a chemical intermediate for most fluorine-containing compounds. Significant direct uses of HF include pickling (cleaning) of steel, cracking of alkanes in the petrochemical industry, and etching of glass.
One third of HF (one sixth of mined fluorine) is used to make synthetic cryolite ( sodium hexafluoroaluminate) and aluminium trifluoride. These compounds are used in the electrolysis of aluminium. About 23 kg (51 lb) are required for every tonne of aluminium. These compounds are also used as a flux for glass.
Fluorosilicates are the next most significant inorganic fluorides formed from HF. The most common one, that of sodium, is used for water fluoridation, as an intermediate for synthetic cryolite and silicon tetrafluoride, and for treatment of effluents in laundries.
MgF2 and, to a lesser extent, other alkaline earth difluorides are specialty optical materials. Magnesium difluoride is widely used as an antireflection coating for spectacles and optical equipment. The compound is also a component in newly devised constructions ( negative index metamaterials) which are the subject of "invisibility" research. The layered structures can curve light around objects.
Other inorganic fluorides made in large quantities include cobalt difluoride (for organofluorine synthesis), nickel difluoride (electronics), lithium fluoride (a flux), sodium fluoride (water fluoridation), potassium fluoride (flux), and ammonium fluoride (various). Sodium and potassium bifluorides are significant to the chemical industry.
Fluorocarbons
Making organic fluorides is the main use for hydrofluoric acid, consuming over 40% of it (over 20% of all mined fluorite). Within organofluorides, refrigerant gases are still the dominant segment, consuming about 80% of HF. Even though chlorofluorocarbons are widely banned, the replacement refrigerants are often other fluorinated molecules. Fluoropolymers are less than one quarter the size of refrigerant gases in terms of fluorine usage, but are growing faster. Fluorosurfactants are a small segment in mass but are significant economically because of very high prices.
Refrigerant gases
Traditionally chlorofluorocarbons (CFCs) were the predominant fluorinated organic chemical. CFCs are identified by a system of numbering that explains the amount of fluorine, chlorine, carbon and hydrogen in the molecules. The term Freon has been colloquially used for CFCs and similar halogenated molecules, though strictly speaking this is just a DuPont brand name, and many other producers exist. Brand neutral terminology is to use "R" as the prefix. Prominent CFCs included R-11 ( trichlorofluoromethane), R-12 ( dichlorodifluoromethane), and R-114 ( 1,2-dichlorotetrafluoroethane).
Production of CFCs grew strongly through the 1980s, primarily for refrigeration and air conditioning but also for propellants and solvents. Since the end use of these materials is banned in most countries, this industry has shrunk dramatically. By the early 21st century, production of CFCs was less than 10% of the mid-1980s peak, with remaining use primarily as an intermediate for other chemicals. The banning of CFCs initially depressed the overall demand for fluorite but 21st century production of the source mineral has recovered to 1980s levels.
Hydrochlorofluorocarbons (HCFCs) and hydrofluorocarbons (HFCs) now serve as replacements for CFC refrigerants; few were commercially manufactured before 1990. Currently more than 90% of fluorine used for organics goes into these two classes (in about equal amounts). Prominent HCFCs include R-22 ( chlorodifluoromethane) and R-141b ( 1,1-dichloro-1-fluoroethane). The main HFC is R-134a ( 1,1,1,2-tetrafluoroethane).
A bromofluoroalkane, "Halon" ( bromotrifluoromethane) is still widely used in ship and aircraft gaseous fire suppression systems. Because Halon production has been banned since 1994, systems are dependent on the pre-ban stores and on recycling.
Fluoropolymers
Fluoropolymers are less than 0.1% of all polymers produced in terms of weight. They are more expensive and have higher growth rates than average polymers. As of about 2006–2007, estimates of the global fluoropolymer production varied from over 100,000 to 180,000 metric tons per year. Yearly revenue estimates ranged from over $2.5 billion to over $3.5 billion.
PTFE (polytetrafluoroethylene) is 60–80% of the world's fluoropolymer production on a weight basis. The term Teflon is sometimes used generically for the substance, but is a DuPont brand name—other PTFE producers exist and DuPont sometimes uses the Teflon brand for other materials. PTFE gets its fluorine without the need for fluorine gas: chloroform (trichloromethane) is treated with HF to make chlorodifluoromethane (R-22, an HFC); this chemical when heated makes tetrafluoroethylene (abbreviated TFE), the starting point for PTFE.
The largest application for PTFE is in electrical insulation. It is an excellent dielectric and very chemically stable. It is also used extensively in the chemical process industry where corrosion resistance is needed: in coating pipes, in tubing, and gaskets. Another major use is architectural fabric (PTFE-coated fibreglass cloth used for stadium roofs and such). The major consumer application is non-stick cookware.
When stretched with a jerk, PTFE films makes a fine-pored membrane: expanded PTFE ( ePTFE). The term "Gore-tex" is sometimes used generically for this material, but that is a specific brand name. W.L. Gore is not the only producer of ePTFE and furthermore "Gore-tex" often refers to more complicated multi-layer membranes or laminated fabrics. ePTFE is used in rainwear, protective apparel and liquids and gas filters. PTFE can also be formed into fibers which are used in pump packing (seals) and bag house filters for industries with corrosive exhausts.
Other fluoropolymers tend to have similar properties to PTFE—high chemical resistance and good dielectric properties—which leads to use in the chemical process industry and electrical insulation. They are easier to work with (to form into complex shapes), but are more expensive than PTFE and have lower thermal stability. Fluorinated ethylene propylene (FEP) is the second most produced fluoropolymer. Films from two different fluoropolymers serve as glass-replacements in solar cells.
Fluorinated ionomers are expensive, chemically resistant materials used as membranes in certain electrochemical cells. Nafion, developed in the 1960s, was the first example and remains the most prominent material in the class. The initial Nafion application was as a fuel cell material in spacecraft. Since then, the material has been transforming the 55 million ton per year chloralkali industry; it is replacing hazardous mercury-based cells with membrane cells that are also more energy efficient. While older technology plants continue to run, new plants typically use membrane cells. By 2002, more than a third of the global capacity for the industry was membrane-cell based. Recently, the fuel cell application has reemerged; significant research is being conducted and investments made related to getting proton exchange membrane (PEM) fuel cells into vehicles.
Fluoroelastomers are rubber-like substances that are composed of crosslinked mixtures of fluoropolymers. Viton is a prominent example. Chemical-resistant O-rings are the primary application. Fluoroelastomers tend to be more stiff than conventional elastomers, but with superior chemical and heat resistance.
Surfactants
Fluorinated surfactants are small organofluorine molecules, principally used in DWR (durable water repellent). Fluorosurfactants form a large market, over $1 billion per year as of 2006. Scotchgard is a prominent brand, with over $300 million revenue in 2000. Fluorosurfactants are expensive chemicals, comparable to pharmaceutical chemicals: $200–2000 per kilogram ($90–900 per pound).
Fluorosurfactants make a very small part of the overall surfactant market, most of which is hydrocarbon based and much cheaper. Some potential applications (e.g. low cost paints) are unable to use fluorosurfactants because of the price impact of compounding in even small amounts of fluorosurfactant. Use in paints was only about $100 million as of 2006.
DWR is a finish (very thin coating) put on fabrics that makes them lightly rain resistant, that makes water bead. First developed in the 1950s, fluorosurfactants were 90% of the DWR industry by 1990. DWR is used with garment fabrics, carpeting, and food packaging. DWR is applied to fabrics by "dip-squeeze-dry" (immersion in DWR-water bath, pressing water out, and then drying).
Fluorine gas
For countries with available data (free-market countries), about 17,000 metric tons of fluorine are produced per year by 11 companies, all G7-resident. Fluorine is relatively inexpensive, costing about US$5–8 per kilogram ($2–4 per pound) when sold as uranium hexafluoride or sulfur hexafluoride. Because of difficulties in storage and handling, the price of pure fluorine gas is much higher. Processes demanding large amounts of fluorine gas generally vertically integrate and produce the gas onsite for direct use.
The largest application for elemental fluorine is the preparation of uranium hexafluoride, which is used in the production of nuclear fuels. To obtain the compound, uranium dioxide is first treated with hydrofluoric acid, to produce uranium tetrafluoride. This compound is then further fluorinated by direct exposure to fluorine gas to make the hexafluoride. Fluorine's monoisotopic natural occurrence makes it useful in uranium enrichment, because uranium hexafluoride molecules will differ in mass only because of mass differences between uranium-235 and uranium-238. These mass differences are used to separate uranium-235 and uranium-238 via diffusion and centrifugation. Up to 7,000 tons per year of fluorine gas are used for this application.
The second largest application for fluorine gas is sulfur hexafluoride, which is used as a dielectric medium in high voltage switching stations. SF6 gas has a much higher dielectric strength than air. It is extremely inert and, compared to oil-filled switchgear, has no PCB (a hazardous chemical). Sulfur hexafluoride is also used in soundproof windows, in the electronics industry, as well as niche medical and military applications. The compound can be made without using fluorine gas, but the reaction between pure sulfur and pure fluorine gas, first developed by Moissan, remains the commercial practice. About 6,000 tons per year of fluorine gas are consumed.
Several compounds made from elemental fluorine serve the electronics industry. Rhenium and tungsten hexafluorides are used for chemical vapor deposition of thin metal films onto semiconductors. Tetrafluoromethane, is used for plasma etching in semiconductor manufacturing, flat panel display production, and microelectromechanical systems fabrication. Nitrogen trifluoride is increasingly used for cleaning equipment at display manufacturing plants. Elemental fluorine, itself, is used sometimes for cleaning equipment.
For making niche organofluorines and fluorine-containing pharmaceuticals, direct fluorination is usually too hard to control. Preparation of intermediate strength fluorinators from fluorine gas solves this problem. The halogen fluorides ClF3, BrF3, and IF5 provide gentler fluorination, with a series of strengths. They are also easier to handle. Sulfur tetrafluoride is used particularly for making fluorinated pharmacueticals.
United States and Soviet space scientists in the early 1960s studied elemental fluorine as a possible rocket propellant because of the higher specific impulse generated when fluorine replaced oxygen in combustion. The experiments failed because fluorine proved difficult to handle, and its combustion product (typically hydrogen fluoride) was extremely toxic and corrosive.
Production of fluorine gas
Commercial producers of fluorine gas continue to use the method of electrolysis pioneered by Moissan, with some modifications in the cell design. Owing to the gas's corrosiveness, special containment materials and handling precautions are required. Chemical routes to the elemental form were published in 1986.
Electrolytic synthesis
Several thousand tons of elemental fluorine are produced annually by electrolysis of potassium bifluoride in hydrogen fluoride. Potassium bifluoride forms spontaneously from potassium fluoride and the hydrogen fluoride:
- HF + KF → KHF2
A mixture with the approximate composition KF•2HF melts at 70 °C (158 °F) and is electrolyzed between 70 °C and 130 °C (160–265 °F). Potassium bifluoride increases the electrical conductivity of the solution and provides the bifluoride anion, which releases fluorine at the anode (negative part of the cell). If HF alone is electrolyzed, hydrogen forms at the cathode (positive part of the cell) and the fluoride ions remain in solution. After electrolysis, potassium fluoride remains in solution.
- 2 HF2– → H2↑ + F2↑ + 2 F–
The modern version of the process uses steel containers as cathodes, while blocks of carbon are used as anodes. The carbon electrodes are similar to those used in the electrolysis of aluminium. An earlier version of fluorine production process, by Moissan, uses platinum group metal electrodes and carved fluorite containers. The voltage for the electrolysis is between 8 and 12 volts.
Handling
Pure fluorine gas may be stored in steel cylinders where the inside surface is passivated by a metal fluoride layer that resists further attack. Passivated steel will withstand fluorine provided the temperature is kept below 200 °C (400 °F). Above that temperature, nickel is required. Regulator valves are made of nickel. Fluorine piping is generally made of nickel or Monel (nickel-copper alloy). Care must be taken to passivate all surfaces frequently and to exclude any water or greases. In the laboratory, fluorine gas can be used in glass tubing provided the pressure is low and moisture is excluded, although some sources recommend systems made of nickel, monel, and PTFE.
Chemical routes
In 1986, when preparing for a conference to celebrate the 100th anniversary of the discovery of fluorine, Karl Christe discovered a purely chemical preparation of fluorine gas; however, he stated in his work that the basics were known 50 years before the actual reaction. The main idea is that some metal fluoride anions do not have a neutral counterpart (or those are very unstable) and their acidifying would result in chemical oxidation, rather than formation of the expected molecules. Christe lists the following reactions as a possible way:
- 2 KMnO4 + 2 KF + 10 HF + 3 H2O2 → 2 K2MnF6 + 8 H2O + 3 O2↑
- 2 K2MnF6 + 4 SbF5 → 4 KSbF6 + 2 MnF3 + F2↑
This synthetic route is a rare chemical preparation of elemental fluorine, a reaction not previously thought possible.
Biological aspects
Fluoride is not considered an essential mineral element for mammals and humans. Small amounts of fluoride may be beneficial for bone strength, but this is an issue only in the formulation of artificial diets. Food and drinking water typically contain at least small amounts of fluorides, which are naturally present.
Several other biological aspects of fluorine exist. Fluoride is widely used for prevention of dental cavities. In pharmaceuticals and agrichemicals, fluorine sees increasing use in new molecules. Poisons containing fluorine are well known for killing insects and rodents and the very few organisms that incorporate fluorine in their biochemistry do so to make natural poisons. Both radioactive and natural fluorine isotopes are important in respective scanning applications. Oxygen-carrying perfluorocarbons present a possibility for human liquid breathing.
Dental care
Fluoride ions in contact with teeth have been long thought to limit cavities by turning the forming the hydroxyapatite of teeth into less soluble fluorapatite. The more up-to-date studies show no difference in caries levels between teeth with enamel fluoridated to different degrees, while low levels of fluoride in plaque fluid and saliva definitely do help to fight the early caries. The process of fluoride absorption works only by direct contact ( topical treatment). Fluoride ions that are swallowed do not benefit the teeth.
Water fluoridation is the controlled addition of fluoride to a public water supply to reduce tooth decay. Its use began in the 1940s, following studies of children in a region where water is naturally fluoridated. It is now used for about two-thirds of the U.S. population on public water systems and for about 5.7% of people worldwide. Although the best available evidence shows no association with adverse effects other than fluorosis (dental and, in worse cases, skeletal), most of which is mild, water fluoridation has been contentious for ethical, safety, and efficacy reasons, and opposition to water fluoridation exists despite its support by public health organizations. The benefits of water fluoridation have lessened recently, presumably because of the availability of fluoride in other forms, but are still measurable, particularly for low income groups. Systematic reviews in 2000 and 2007 showed significant reduction of cavities in children associated with water fluoridation.
Sodium fluoride, tin difluoride, and, most commonly, sodium monofluorophosphate, are used in toothpaste. In 1955, the first fluoride toothpaste was introduced, in the United States. Now, almost all toothpaste in developed countries is fluoridated. For example, 95% of European toothpaste contains fluoride. Gels and foams are often advised for special patient groups, particularly those undergoing radiation therapy to the head (cancer patients). The patient receives a four-minute application of a high amount of fluoride. Varnishes exist that perform a similar function, but are more quickly applied. Fluoride is also contained in prescription and non-prescription mouthwashes and is a trace component of foods manufactured from fluoridated water supplies.
Pharmaceuticals
Several important pharmaceuticals contain fluorine. Of commercialized drugs, already 20% contain fluorine, as even a single atom of it improves the chemical reactivity of the drug molecule used.
Because of the considerable stability of the carbon-fluorine bond, many drugs are fluorinated to delay their metabolism (turning into another molecule; the chemical process behind this is how the drugs work). This prolongs their half-lives and allows for longer times between dosing and activation. For example, an aromatic ring may add to prevent the metabolism of a drug, but this presents a safety problem, because some aromatic compounds are metabolized in the body into poisonous epoxides by the organism's native enzymes. Substituting a fluorine into a para position, however, protects the aromatic ring and prevents the epoxide from being produced.
Adding fluorine to biologically active organics increases their lipophilicity (ability to dissolve in fats), because the carbon–fluorine bond is even more hydrophobic than the carbon–hydrogen bond. This effect often increases a drug's bioavailability because of increased cell membrane penetration. Although the potential of the fluorine to be released as a fluoride leaving group depends on its position in the molecule, organofluorides are generally very stable, since the carbon–fluorine bond is strong.
Fluorines also find their uses in common mineralocorticoids, a class of drugs that increase the blood pressure. Adding a fluorine increases both its medical power and anti-inflammatory effects. Fluorine-containing fludrocortisone is one of the most common such drugs. Dexamethasone and triamcinolone, which are among the most potent of the related synthetic corticosteroids class of drugs, contain fluorine as well.
Several inhaled general anesthetic agents, including the most commonly used inhaled agents, also contain fluorine. Examples include sevoflurane, desflurane, and isoflurane, which are hydrofluorocarbon derivatives.
Many SSRI antidepressants are fluorinated organics, including citalopram, escitalopram, and fluoxetine. Fluoroquinolones are a commonly used family of broad-spectrum antibiotics.
![]() |
![]() |
![]() |
![]() |
---|---|---|---|
Lipitor (atorvastatin) | 5-FU (fluorouracil) | Florinef (fludrocortisone) | Isoflurane |
Agrichemicals and natural poisons
An estimated 30% of agrichemical compounds contain fluorine. Most of them are poisons, but a few stimulate the growth instead. The further fluorine agrichemicals usage is said to rely on improvement of synthesis reaction (lowering the costs raised by fluorine content) and level of greener chemistry consideration (fluorochemicals are more environment-friendly).
Synthetic sodium fluoroacetate has been used as an insecticide but is especially effective against mammalian pests. The name "1080" refers to the catalogue number of the poison, which became its brand name. Fluoroacetate is similar to acetate, which has a pivotal role in the Krebs cycle (a key part of cell metabolism). Fluoroacetate halts the cycle and causes cells to be deprived of energy. Several other insecticides contain sodium fluoride, which is much less toxic than fluoroacetate. Currently the compound is banned. Another important agrichemcial is Trifluralin. It was once very important (for example, in 1998 over a half of U.S. cotton field area was coated with the chemical); however, its suspected carcinogenic properties caused some Northern European countries to ban it in 1993. Currently, the whole European Union has it banned, although there was a case intended to cancel the decision.
The currently used agrichemicals utilize another tactic: Instead of being poisonous themselves, they penetrate into the metabolism not to affect it directly, but to transform into actually poisonous compounds. For example, insects fed 29-fluorostigmasterol produce the fluoroacetates from it. If a fluorine is transferred to a body cell, it blocks metabolism at the position occupied.
Biologically synthesized organofluorines have been found in microorganisms and plants, but not in animals. The most common example is fluoroacetate, with an active poison molecule identical to commercial "1080". It is used as a defense against herbivores by at least 40 green plants in Australia, Brazil, and Africa; other biologically synthesized organofluorines include ω-fluoro fatty acids, fluoroacetone, and 2-fluorocitrate. In bacteria, the enzyme adenosyl-fluoride synthase, which makes the carbon–fluorine bond, was isolated. The discovery was touted as possibly leading to biological routes for organofluorine synthesis.
Scanning
Compounds containing fluorine-18, a radioactive isotope that emits positrons, are often used in positron emission tomography (PET) scanning, because the isotope's half-life of about 110 minutes is long by positron-emitter standards. One such radiopharmaceutical is 2-deoxy-2-(18F)fluoro-D-glucose (generically referred to as fludeoxyglucose), commonly abbreviated as 18F-FDG, or simply FDG. In PET imaging, FDG can be used for asessing glucose metabolism in the brain and for imaging cancer tumors. After injection into the blood, FDG is taken up by "FDG-avid" tissues with a high need for glucose, such as the brain and most types of malignant tumors. Tomography, often assisted by a computer to form a PET/CT (CT stands for "computer tomography") machine, can then be used to diagnose or monitor treatment of cancers; especially Hodgkin's lymphoma, lung cancer, and breast cancer.
Natural fluorine is monoisotopic, consisting solely of fluorine-19. Fluorine compounds are highly amenable to nuclear magnetic resonance (NMR), because fluorine-19 has a nuclear spin of ½, a high nuclear magnetic moment, and a high magnetogyric ratio. Fluorine compounds typically have a fast NMR relaxation, which enables the use of fast averaging to obtain a signal-to-noise ratio similar to hydrogen-1 NMR spectra. Fluorine-19 is commonly used in NMR study of metabolism, protein structures and conformational changes. In addition, inert fluorinated gases have the potential to be a cheap and efficient tool for imaging lung ventilation.
Oxygen transport research
Liquid fluorocarbons have a very high capacity for holding gas in solution. They can hold more oxygen or carbon dioxide than blood does. For that reason, they have attracted ongoing interest related to the possibility of artificial blood or of liquid breathing.
Blood substitutes are the subject of research because the demand for blood transfusions grows faster than donations. In some scenarios, artificial blood may be more convenient or safe. Because fluorocarbons do not normally mix with water, they must be mixed into emulsions (small droplets of perfluorocarbon suspended in water) to be used as blood. One such product, Oxycyte, has been through initial clinical trials.
Possible medical uses of liquid breathing (which uses pure perflurocarbon liquid, not a water emulsion) involve assistance for premature babies or for burn victims (because the normal lung function is compromised). Both partial filling of the lungs and complete filling of the lungs have been considered, although only the former has any significant tests in humans. Several animal tests have been performed and some human partial liquid ventilation trials. One effort, by Alliance Pharmaceuticals, reached clinical trials but was abandoned because of insufficient advantage compared to other therapies.
Nanocrystals represent a possible method of delivering water or fat soluble drugs within a perfluorochemical fluid. The particles usage is being developed to help treat babies with damaged lungs.
Other posited applications include deep sea diving and space travel, applications that would both require total liquid ventilation, not partial ventilation. The 1989 film The Abyss showed a fictional use of perfluorocarbon for human diving but also filmed a real rat surviving while cooled and immersed in perfluorocarbon. (See also list of fictional treatments of perfluorocarbon breathing.)
Hazards
Fluorine gas and fluoride ion
Elemental fluorine is highly toxic. Above a concentration of 25 ppm, fluorine causes significant irritation while attacking the eyes, respiratory tract, lungs, liver and kidneys. At a concentration of 100 ppm, human eyes and noses are seriously damaged.
Soluble fluorides are moderately toxic. For sodium fluoride, the lethal dose for adults is 5–10 g, which is equivalent to 32–64 mg of elemental fluoride per kilogram of body weight. The dose that may lead to adverse health effects is about one fifth the lethal dose. Chronic excess fluoride consumption can lead to skeletal fluorosis, a disease of the bones that affects millions in Asia and Africa.
The fluoride ion is readily absorbed by the stomach and intestines. Ingested fluoride forms hydrofluoric acid in the stomach. In this form, fluoride crosses cell membranes and then binds with calcium and interfere with various enzymes. Fluoride is excreted through urine. Fluoride exposure limits are based on urine testing which has determined the human body's capacity for ridding itself of fluoride.
Historically, most cases of fluoride poisoning have been caused by accidental ingestion of insecticides containing inorganic fluoride, Currently, most calls to poison control centers for possible fluoride poisoning come from the ingestion of fluoride-containing toothpaste. Malfunction of water fluoridation equipment has occurred several times, including an Alaskan incident, which affected nearly 300 people and killed one.
Hydrofluoric acid
Hydrofluoric acid is a contact poison and must be handled with extreme care, far beyond that accorded to other mineral acids. Owing to its lesser chemical dissociation in water (remaining a neutral molecule), hydrogen fluoride penetrates tissue more quickly than typical acids. Poisoning can occur readily through exposure of skin or eyes, or when inhaled or swallowed. Symptoms of exposure to hydrofluoric acid may not be immediately evident, with 8-hour delay for 50% HF and up to 24-hour if the concentration is smaller. Hydrogen fluoride interferes with nerve function, meaning that burns may not initially be painful. Accidental exposures can go unnoticed, delaying treatment and increasing the extent and seriousness of the injury. If the burn has been initially noticed, then HF should be washed off with a forceful stream of water for ten to fifteen minutes, to prevent its further penetration into the body. Clothing used by the person burned may also exhibit danger.
Once in the blood, hydrogen fluoride reacts with calcium and magnesium, resulting in electrolyte unbalance, cardiac arrhythmia, and potentially, death. Formation of insoluble calcium fluoride possibly causes both a fall in calcium serum and the strong pain associated with tissue toxicity. In some cases, exposures can lead to hypocalcemia. Burns with areas larger than 160 cm2 (25 in2) can cause serious systemic toxicity from interference with blood and tissue calcium levels.
Hydrofluoric acid exposure is often treated with calcium gluconate, a source of Ca2+ that binds with the fluoride ions. Skin burns can be treated with a water wash and 2.5% calcium gluconate gel or special rinsing solutions. However, because HF is absorbed, medical treatment is necessary; sometimes amputation may be required.
Environmental concerns
Atmosphere
Chlorofluorocarbons (CFCs) and bromofluorocarbons (BFCs) have been strictly regulated via a series of international agreements, the Montreal Protocol, because they deplete the ozone layer. It is the chlorine and bromine from these molecules that cause harm, not fluorine. Because of the inherent stability of these fully halogenated molecules (which makes them so nonflammable and useful), they are able to reach the upper reaches of the atmosphere, before decomposing, and then release chlorine and bromine to attack the ozone at those altitudes. Predictions are that generations will be required, even after the CFC ban, for these molecules to leave the atmosphere and for the ozone layer to recover. Early indications are that the CFC ban is working—ozone depletion has stopped and recovery is underway.
Hydrochlorofluorocarbons (HCFCs) are current replacements for CFCs; HCFCs have about one tenth the ozone damaging potential (ODP) of CFCs. They were originally scheduled for elimination by 2030 in developed nations (2040 in undeveloped). In 2007, a new treaty was signed by almost all nations to move that phaseout up by ten years because HFCs, which have no chlorine and thus zero ODP, are available. Meanwhile, individual HCFCs with the highest anti-ozone depleting potential are being phased out first. For example, in 2003, HCFC-141b was phased out in the U.S. by Environmental Protection Agency regulation. Many of the other HCFCs are now being produced at a fraction of their previous production rates.
Fluorocarbon gases of all sorts (CFCs, HFCs, etc.) are greenhouse gases about 4,000 to 10,000 times as potent as carbon dioxide. Sulfur hexafluoride exhibits an even stronger effect, about 20,000 times the global warming potential of carbon dioxide.
Biopersistance
Because of the strength of the carbon–fluorine bond, organofluorines endure in the environment. Perfluorooctanoic acid (PFOA) and perfluorooctanesulfonic acid (PFOS), used in waterproofing sprays, are persistent global contaminants. Trace quantities of these substances have been detected worldwide, from polar bears in the Arctic to the global human population. One study indicates that PFOS levels in wildlife are starting to go down because of the recent reduced production of that chemical.
PFOA's tissue distribution in humans is unknown, but studies in rats suggest it is likely to be present primarily in the liver, kidney, and blood. In the body, PFOA binds to a protein, serum albumin; it has been detected in breast milk and the blood of newborns. PFOA is not metabolized by the body, but is excreted by the kidneys.
The potential health effects of PFOA are unclear. Unlike chlorinated hydrocarbons, PFOA is not lipophilic (stored in fat), nor is it genotoxic (damaging genes). While both PFOA and PFOS cause cancer in high quantities in animals, studies on exposed humans have not been able to prove an impact at current exposures. Bottlenose dolphins have some of the highest PFOS concentrations of any wildlife studied; one study suggests an impact on their immune systems.
Because biological systems do not metabolize fluorinated molecules easily, fluorinated pharmaceuticals (often antibiotics and antidepressants) are among the major fluorinated organics found in treated city sewage and wastewater. Fluorine-containing agrichemicals are measurable in farmland runoff and nearby rivers.
Compounds
Fluorine's only common oxidation state is −1. With other atoms, fluorine forms either polar covalent bonds or ionic bonds. Most frequently, covalent bonds involving fluorine atoms are single bonds, although at least two examples of a higher order bond exist. Fluoride may act as a bridging ligand between two metals in some complex molecules. Molecules containing fluorine may also exhibit hydrogen bonding. Fluorine has a rich chemistry including inorganic compounds formed with hydrogen, metals, nonmetals, and even noble gases; as well as a diverse set of organic compounds.
Inorganic
Hydrogen fluoride
Fluorine combines with hydrogen to make a compound (HF) called hydrogen fluoride or, especially in the context of water solutions, hydrofluoric acid. The H-F bond type is one of the few capable of hydrogen bonding (creating extra clustering associations with similar molecules). This influences various peculiar aspects of hydrogen fluoride's properties. In some ways the substance behaves more like water, also very prone to hydrogen bonding, than one of the other hydrogen halides, such as HCl.
Hydrogen bonding amongst HF molecules gives rise to high viscosity in the liquid phase and lower than expected pressure in the gas phase. Hydrogen fluoride does not boil until 20 °C in contrast to the heavier hydrogen halides which boil between −85 °C and −35 °C (−120–30 °F). HF is fully miscible with water (will dissolve in any proportion), while the other hydrogen halides have large solubility gaps with water. Hydrogen fluoride and water also form several compounds in the solid state, most notably a 1:1 compound that does not melt until −40 °C (−40 °F), which is 44 degrees Celsius (79 degrees Fahrenheit) above the melting point of pure HF.
Unlike other hydrohalic acids, such as hydrochloric acid, hydrogen fluoride is only a weak acid in water solution, with acid dissociation constant (pKa) equal to 3.19. HF's weakness as an aqueous acid is paradoxical considering how polar the HF bond is, much more so than the bond in HCl, HBr, or HI. The explanation for the behaviour is complicated, having to do with various cluster-forming tendencies of HF, water, and fluoride ion, as well as thermodynamic issues. At great concentrations, a property called homoconjugation is revealed. HF begins to accept fluoride ions, forming the polyatomic ions (such as bifluoride, HF−
2) and protons, thus greatly increasing the acidity of the compound. Hydrofluoric acid is also the strongest of the hydrohalic acids in acetic acid and similar solvents. Its hidden acidity potential is also revealed by the fact it protonates acids like hydrochloric, sulfuric, or nitric. Despite its weakness, hydrofluoric acid is very corrosive, even attacking glass (hydrated only).
Dry hydrogen fluoride dissolves low-valent metal fluorides readily. Several molecular fluorides also dissolve in HF. Many proteins and carbohydrates can be dissolved in dry HF and can be recovered from it. Most non-fluoride inorganic chemicals react with HF rather than dissolving.
Metal fluorides
Metal fluorides have similarities with other metal halides but are more ionic. In many respects, metal fluorides differ from other metal halides (chlorides, bromides, iodides), very similar to each other. Instead, fluorides are more similar to oxides, often having similar bonding and crystal structures.
The metal fluorides show broad trends based on the charge of the metal. Metals in an oxidation state of +3 or lower tend to form ionic, refractory fluorides. Metals charged +5 or higher tend to form covalently bonded fluorides, polymers or discrete molecules, and are more volatile. (The tetrafluorides are a transition zone.) The bonding variations mean that metal fluorides can be solids, liquids, or gases at room temperature.
The solubility of fluorides varies greatly but tends to decrease as the charge on the metal ion increases. Dissolved fluorides produce basic solutions. (F- is a weak base because HF is a weak acid.) Like hydroxides, fluorides may be viewed as basic, amphoteric, and acidic, with the acidity property generally increasing with the oxidation state of the metal; a fluoride is a much weaker base than a hydroxide, though.
Low oxidation states
The alkali metals form monofluorides. All are soluble and have the sodium chloride (rock salt) structure, which is also adopted by some alkaline earth oxides such as CaO. Because the fluoride anion is highly basic, many alkali metal fluorides form bifluorides with the formula MHF2. They also give off the fluoride easily when reacting with an acid. Among other monofluorides, only silver(I) and thallium(I) fluorides are well-characterized. Both are very soluble, unlike the other halides of those metals. Another silver fluoride is from this point a "half-fluoride" (a subfluoride, compound containing less than normal saturation with fluorine), formulated as Ag2F. This compound has been described as having F- and unusual "Ag½+" centers.
Unlike the monofluorides, the difluorides may be either soluble or insoluble. Several transition metal difluorides, such as those of copper(II) and nickel(II), are soluble. The alkaline earth metals form difluorides that are insoluble. In contrast, the alkaline earth chlorides are readily soluble. The insolubility of alkaline earth fluorides comes from the high negative lattice energy of the common in chemistry fluorite structure (adopted by several metal dioxides such as CeO2, UO2, ThO2, etc.), so that it is more favorable for the solid to stay solid rather than dissolve, so they are insoluble (see the table, notice the omitted minus signs). However, beryllium fluoride shows striking differences from other alkaline earth fluorides, just is beryllium different than other alkaline earth metals (tending to bond covalently, so BeF2 shows a significant covalent character). The compounds has many similarities to SiO2 (quartz): a mostly covalently bonded network solid, glasses formation (are difficult to crystallize), the same room temperature crystal structure when crystalline, etc. It is very soluble in water, unlike the other alkaline earths.
Many metals form trifluorides, such as iron, bismuth, the rare earth elements, and the metals in the aluminium column of the periodic table. At this point, the ionicity of the fluorides begins to reduce, and acidity begins to grow, even though the compounds are still ionic solids, mainly weakly basic. In many cases, while the trifluorides are still ionic, other trihalides may be volatile (for example, aluminium or gold). No trifluoride is soluble in water, but several are soluble in other solvents.
The tetrafluorides show a mixture of ionic and covalent bonding: Zirconium, hafnium, plus many of the actinides form square-antiprismatic ionic, high-melting tetrafluorides. Titanium, niobium, and vanadium tetrafluorides are polymeric, with melting or decomposition points below 400 °C (vanadium's may also disproportionate at 100–120 °C to the trifluoride and the pentafluoride). Many transition metals tetrafluorides show unusual structures intermediate between ionic and monomeric covalent (notably iridium tetrafluoride featuring both shared and unshared fluorines). They typically have low melting points (osmium tetrafluoride's is, for example, 230 °C) or are of low stability (manganese tetrafluoride decomposes even at room temperature). Germanium tetrafluoride forms tetrahedral molecules and is a gas at room temperature.
High oxidation states
Metal penta- and higher fluorides are all covalently bonded and volatile. This behaviour contrasts with the corresponding oxides. Oxygen is a weaker oxidant and inherently more likely to form covalent bonds, but it only forms molecules with five metals ( manganese heptoxide, technetium heptoxide, ruthenium tetroxide, osmium tetroxide, and iridium tetroxide). Fluorine forms molecules with fifteen metals because its small size and single charge as an ion allows surrounding metal atoms with more fluorines than oxygen can.
Bismuth's highest fluoride is a volatile pentafluoride that is a powerful fluorinating agent. In the solid state, it is polymeric, consisting of linear chains of octahedra, sharing axial fluorides. Pentavalent bismuth behaves as an acid and forms hexafluorobismuthate, [BiF6]−, upon reaction with a fluoride donor, either strong (such as NaF) or not (such as XeF4), thus being an acid. Niobium and tantalum pentafluorides, while thermally stable, are also strong acids and thus hydrolyze easily. They are truly molecular, forming tetrameric molecules. Many metals that form higher fluorides also can form pentafluorides, but they may be unstable. For example, osmium and ruthenium pentafluorides, while also polymeric, have a different structure, and are of low stability (hydrolyze easily, are not of the highest oxidation state, nor a stable one).
The metals that make well-characterized hexafluorides include nine metals in the centre of the periodic table and three actinides. At room temperature, tungsten hexafluoride is a gas, molybdenum hexafluoride and rhenium hexafluoride are liquids, and the rest are volatile solids. Metal hexafluorides are oxidants because of their tendency to get reduced: for example, platinum hexafluoride was the first compound to oxidize molecular oxygen and xenon. The least unstable hexafluoride is that of osmium. Polonium also forms a hexafluoride, but it is understudied.
Rhenium is the only metal known to bond with seven fluorides in charge-neutral metal compound, which is the charged-ligands number record. Rhenium heptafluoride can be synthesized from the elements, and is relatively stable against heat. Chemically, it may serve as a non-oxidizing acid to form the corresponding anion, ReF−
8, or with stronger acids as a base to form a cation, ReF+
6. The molecule adopts a pentagonal bipyramid molecular geometry (expected to be shared with the currently unknown but perhaps possible iridium heptafluoride (report of synthesis is being prepared), technetium heptafluoride, and osmium heptafluoride).
Nonmetal fluorides
The nonmetal binary fluorides are volatile compounds. They show a great difference between period 2 and other fluorides. For instance, period 2 elements elements fluorides never exceed the octet in their atoms. (Boron is an exception due to its specific position in the periodic table.) Lower-period elements, however, may form hypervalent molecules, such as phosphorus pentafluoride or sulfur hexafluoride. The reactivity of such species varies greatly—sulfur hexafluoride is inert, while chlorine trifluoride is extremely reactive—but there are some trends based on periodic table locations.
Boron trifluoride is a planar molecule. It has only six electrons around the central boron atom (and thus an incomplete octet), but it readily accepts a Lewis base, forming adducts with lone-pair-containing molecules or ions such as ammonia or another fluoride ion which can donate two more electrons to complete the octet. Boron monofluoride is an unstable molecule with an unusual (higher than single) bond to fluorine. The bond order has been described as 1.4 (intermediate between a single and double bond). It is isoelectronic with N2.
Silicon tetrafluoride, similar to carbon tetrafluoride and germanium tetrafluoride, adopts a molecular tetrahedral structure. SiF4 is stable against heating or electric spark, but reacts with water (even moist air), metals, and alkalies, thus demonstrating weak acidic character. Reactions with organomagnesium compounds, alcohols, amines, and ammonia yield adduction compounds. Fluorosilicic acid, a derivative of SiF4, is a strong acid in aqueous solution (the anhydrous form does not exist).
Pnictogens (nitrogen's periodic table column) show very similar trends in reactivity and acidity of the highest fluorides (pentafluorides) and most common ones (trifluorides), with the said property increasing down the group: NF3 is stable against hydrolysis, PF3 hydrolyzes very slowly in moist air, while AsF3 completely hydrolyzes. SbF3 hydrolyzes only partially because of the increasing ionic character of the bond to fluorine. The compounds are weak Lewis bases, with NF3 again being an exception. The pentafluorides of phosphorus and arsenic are much more reactive than their trifluorides; antimony pentafluoride is such a strong acid that it holds the title of the strongest Lewis acid. Nitrogen is not known to form a pentafluoride, although the tetrafluoroammonium cation (NF+
4) features nitrogen in the formal oxidation state of +5. Nitrogen monofluoride is a metastable species that has been observed in laser studies. It is isoelectronic with O2 and like BF, unusually, has a higher bond order than single-bonded fluorine.
The chalcogens (oxygen's periodic table column) are somewhat similar: The tetafluorides are thermally unstable and hydrolyze, and are also ready to use their lone pair to form adducts to other (acidic) fluorides. Sulfur and selenium tetrafluorides are molecular while TeF4 is a polymer. The hexafluorides are the result of direct fluorination of the elements (compare: other hexahalides of the elements do even not exist). They increase in reactivity with atomic number: SF6 is extremely inert, SeF6 is less noble (for example, reacts with ammonia at 200 °C (400 °F)), and TeF6 easily hydrolyzes to give an oxoacid. Oxygen's highest fluoride is oxygen difluoride, but fluorine can theoretically (as of 2012) oxidize it to a uniquely high oxidation state of +4 in the fluorocation: OF+
3.
The well-characterized heavier halogens (chlorine, bromine, and iodine) all form mono-, tri-, and pentafluorides: XF, XF3, and XF5. Of the neutral +7 species, only iodine heptafluoride is known. While chlorine and bromine heptafluorides are not known, the corresponding cations ClF+
6 and BrF+
6, extremely strong oxidizers, are. Astatine is not well-studied, and although there is a report of a non-volatile astatine monofluoride, its existence is debated. Many of the halogen fluorides are powerful fluorinators. Chlorine trifluoride is particularly noteworthy—readily fluorinating asbestos and refractory oxides—and may be even more reactive than chlorine pentafluoride. Used industrially, ClF3 requires special precautions similar to those for fluorine gas because of its corrosiveness and hazards to humans.
Superacids
Several important inorganic acids contain fluorine. They are generally very strong because of the high electronegativity of fluorine. One such acid, fluoroantimonic acid (HSbF6), is the strongest charge-neutral acid known. The dispersion of the charge on the anion affects the acidity of the solvated proton (in form of H2F+): The compound has an extremely low pKa of −28 and is 10 quadrillion (1016) times stronger than pure sulfuric acid. Fluoroantimonic acid is so strong that it protonates otherwise inert compounds like hydrocarbons. Hungarian-American chemist George Olah received the 1994 Nobel Prize in chemistry for investigating such reactions.
Noble gas compounds
The noble gases are generally non-reactive because they have fully filled electronic shells, which are extremely stable. Until the 1960s, no chemical bond with a noble gas was known. In 1962, Neil Bartlett used fluorine-containing platinum hexafluoride to react with xenon. He called the compound he prepared xenon hexafluoroplatinate, but since then the product has been revealed to be mixture of different chemicals. Bartlett probably synthesized a mixture of monofluoroxenyl(II) pentafluoroplatinate, [XeF]+[PtF5]–, monofluoroxenyl(II) undecafluorodiplatinate, [XeF]+[Pt2F11]–, and trifluorodixenyl(II) hexafluoroplatinate, [Xe2F3]+[PtF6]–. Bartlett's fluorination of xenon has been called one of the ten most beautiful experiments in the history of chemistry. Later in 1962, xenon was reported to react directly with fluorine to form the di- and tetrafluorides. Since then, chemists have made extensive efforts to form other noble gas fluorides.
Having started the noble gases–fluorine compounds class, xenon is also the noble gas to have the most well-known such compounds. Its binary compounds include xenon difluoride, xenon tetrafluoride, and xenon hexafluoride, as well as their derivatives. Xenon forms several oxyfluorides, such as xenon oxydifluoride, XeOF2, by reaction of xenon tetrafluoride with water. Its upper neighbour, krypton, is the only other one to form a well-established compound: krypton difluoride. Krypton tetrafluoride was reported in 1963, but was subsequently shown to be a mistaken identification; the compound seems to be very hard to synthesize now (although even the hexafluoride may exist).
In strong accordance with the periodic trends, radon is notably more reactive to oxidizers, including fluorine. It has been shown to readily react with fluorine to form a solid compound, which is generally thought to be radon difluoride. The exact composition is uncertain as the compound is prone to decomposition. Calculations indicate that radon difluoride may be ionic, unlike all other binary noble gas fluorides.
The lighter noble gases (helium through argon) do not form stable binary fluorides. Argon forms no binary fluoride, but reacts in extreme conditions with hydrogen fluoride to form argon fluorohydride; it is the only "stable" argon compound. Helium and neon do not form any stable chemical compounds at all. Helium forms helium fluorohydride; it has been shown to be unstable in gas phase, but there are specific conditions for which it may be stable. Neon, the least reactive element, is not now expected to form a stable compound capable of synthesis.
Ununoctium, the last currently known group 18 element, is predicted to form ununoctium difluoride, UuoF2, and ununoctium tetrafluoride, UuoF4, which is likely to have the tetrahedral molecular geometry. However, only a few atoms of ununoctium have been synthesized, and its chemical properties have not been examined.
Highest oxidation states: fluorine versus oxygen
Elements frequently have their highest oxidation state in the form of a binary fluoride. Several elements show their highest oxidation state only in a few compounds, one of which is the fluoride; and some elements' highest known oxidation state is seen exclusively in a fluoride.
For groups 1–5, 10, 13–16, the highest oxidation states of oxides and fluorides are always equal. Differences are only seen in chromium, groups 7–9, copper, mercury, and the noble gases. Fluorination allows elements to achieve relatively low oxidation states that are, however, hard to achieve. For example, no binary oxide is known for krypton, but krypton difluoride is well-studied. At the same time, very high oxidation states are known for oxygen-based species only. For the previously mentioned volatile oxides, there are no corresponding hepta- or octafluorides. (For example, ruthenium octafluoride is unlikely to be ever synthesized, while ruthenium tetroxide has even found an industrial use.) The main problem that prevents fluorine from forming the highest states in covalent hepta- and octafluorides is that it is hard to attach such a large number of ligands around a single atom; the number of ligands is halved in analogous oxides. However, octafluoride anions, such as the octafluoroiodate (IF−
8), octafluorozirconate (ZrF4−
8), and octafluoroxenate (XeF2−
8) anions are well-known.
The highest oxidation states may be uncommon to everyday life, or even industrial usage. For example, the synthesis of mercury tetrafluoride, the first compound to achieve an oxidation state above +2 for a group 12 element, breaking the filled 5d-shell, again showing the significance of the relativistic effects on the heavy elements, and fueling the debate over whether mercury, cadmium, and zinc are transition metals, occurred at cryogenic temperatures and the compound decomposes at the temperatures of solid nitrogen. More unstable still, the only cobalt(V) species, the CoF+
4 cation, has only been observed in gas phase (with no interactions with other atoms, thus no shown stability in any chemical environment). The reason why such unstable species exist is complicated, yet can be summarized as follows on the example of the hypothesized NF5 molecule: According to the modern calculations, five fluorine atoms and one nitrogen atoms can theoretically arrange themselves in different ways, such as NF3 and F2, NF•
4 and F•, NF5, etc. The NF3+F2 system is of the smallest energy (most stable). However, if a NF5 molecule was synthesized, it would have to go through a high-energy transitional state, from which it could decay into two molecules. But since the transitional state is higher in energy than the hexatomic molecule, the energy difference would need to be added to reach the transitional state and thus allow the decay. This energy is called reaction activation barrier. (The second decay mode has an analogical position.) Thus if little energy was added (low temperatures), then the compound could exist; however, the synthesis is a serious problem (not yet solved).
Organic
Carbon–fluorine chemical bond of the organofluorine compounds is the strongest bond in organic chemistry. Along with the low polarizability of the molecules, these are the most important factors contributing to the great stability of the organofluorines.
The carbon–fluorine bond of the smaller molecules is formed in three principal ways: Fluorine replaces a halogen or hydrogen, or adds across a multiple bond. The direct reaction of hydrocarbons with fluorine gas can be dangerously reactive, so the temperature may need to be lowered even to −150 °C (−240 °F). "Solid fluorine carriers", compounds that can release fluorine upon heating, notably cobalt trifluoride, may be used instead, or hydrogen fluoride. After the reaction, the molecular size is not changed significantly, as the elements have very similar van der Waals radii. Direct fluorination becomes even less important when it comes to organohalogens or unsaturated compounds reactions, or when a prefluorocarbon is desired (then HF-based electrolysis is typically used). In contrast, the fluoropolymers are formed by polymerizing free radicals; other techniques used for hydrocarbon polymers do not work in that way with fluorine.
The range of organofluorine compounds is diverse, reflecting the inherent complexity of organic chemistry. A vast number of small molecules exist with varying amounts of fluorine substitution, as well as many polymers—research into particular areas is driven by the commercial value of applications.
Small molecules
Monofluoroalkanes (alkanes with one hydrogen replaced with fluorine) may be chemically and thermally unstable, yet are soluble in many solvents; but as more fluorines are in instead of hydrogens, the stability increases, while melting and boiling points, and solubility decrease. While the densities and viscosities are increased, the dielectric constants, surface tensions, and refractive indices fall.
Partially fluorinated alkanes are the hydrofluorocarbons (HFCs). Substituting other halogens in combination with fluorine gives rise to chlorofluorocarbons (CFCs) or bromofluorocarbons (BFCs) and the like (if some hydrogen is retained, HCFCs and the like). Properties depend on the number and identity of the halogen atoms. In general, the boiling points are even more elevated by combination of halogen atoms because the varying size and charge of different halogens allows more intermolecular attractions. As with fluorocarbons, chlorofluorocarbons and bromofluorocarbons are not flammable: they do not have carbon–hydrogen bonds to react and released halides quench flames.
When all hydrogens are replaced with fluorine to achieve perfluoroalkanes, a great difference is revealed. Such compounds are extremely stable, and only sodium in liquid ammonia attacks them at standard conditions. They are also very insoluble, with few organic solvents capable of dissolving them.
However, if a perfluorocarbon contains double or triple bonds (perfluoroalkenes or - alkynes), a very reactive towards ligand accepting result, even less stable than corresponding hydrocarbons. Difluoroacetylene, which decomposes even under liquid nitrogen temperatures, is a notable example. If such a molecule is asymmetric, then the more fluorinated carbon is attacked, as it holds positive charge caused by the C–F bonds and is shielded weakly (similarly to that how unsaturated hydrocarbons attacked by HF add hydrogen to the more hydrogen-rich atom per Markovnikov's rule).
Perfluorinated compounds, as opposed to perfluorocarbons, is the term used for molecules that would be perfluorocarbons—only carbon and fluorine atoms—except for having an extra functional group (even though another definition exists). They share most of perfluorocarbon properties (inertness, stability, non-wettingness and insolubility in water and oils, slipperiness, etc.), but may differ because of the functional group properties, although the perfluorocarbon tail differ the group-specific properties as compared to those of hydrocarbon-tailed compounds.
Perfluoroalkanic acids organic acids may also be seen as perfluorinated compounds, with perfluoroalkyl joined to a carboxyl group. As the fluorines are added to the acid, its strength grows: consider acetic acid and its mono-, di-, and trifluoroacetic derivatives and their pKa values (4.74, 2.66, 1.24, and 0.23). This happens because with fluorines, the anion formed after giving the proton off becomes stable, an effect caused by fluorine's great inductive effect. Because of this, the trifluoro derative is 33,800 times stronger an acid than acetic. Similarly, the acidity is greatly increased for other perfluorocarboxyl acids, as well as the amines (here, it makes more sense to talk about elimination of basicity, though).
The perfluoroalkanesulfonic acids are also very notable for their acidity. The sulfonic acid derivative, trifluoromethanesulfonic acid, is comparable in strength to perchloric acid. These compounds lower surface energy; for this reason, they, especially perfluorooctanesulfonic acid (PFOS, formerly the active component in brand "Scotchgard") have found industrial use as surfactants (see above).
If a perfluorinated compound has a fluorinated tail, but also a few non-fluorinated carbons (typically two) near the functional group, it is called a fluorotelomer (such molecules are commercially treated as perfluorinated), but such molecules are more of industrial value than chemical. The chain end may similarly be attached to different functiuonal groups (via the hydrogenized terminal carbon), such as hydroxyl resulting in fluorotelomer alcohols, sulfonate resulting in fluorotelomer sulfonates, etc.
Polymers
Fluoropolymers are similar in many regards with smaller molecules; adding fluorine to a polymer affects the properties in the same manner as in small molecules (increasing chemical stability, melting point, reducing flammability, solubility, etc.). Each fluoropolymer has own characteristic properties, though.
The simplest fluoroplastic is polytetrafluoroethylene (PTFE, DuPont brand Teflon), which is a simple linear chain polymer with the repeating structural unit: –CF2–. PTFE has a backbone of carbons single bonded in a long chain, with all side bonds to fluorines. It contains no hydrogens and can be thought of as the perfluoro analog of polyethylene (structural unit: –CH2–). PTFE has high chemical and thermal stability, as expected for a perfluorocarbon, much stronger than polyethylene. Its resistance to van der Waals forces makes PTFE the only known surface to which a gecko cannot stick. The compound, however, lacks an ability to transform upon melting, which is not a problem for various PTFE deratives, namely FEP ( fluorinated ethylene propylene, with some fluorines replaced with the –CF3 group) or PFA ( perfluoroalkoxy, some fluorines replaced with –OCF3). They share most properties with PTFE, but there are still differences, namely maximum usage temperature (highest for the non-flexible PTFE).
There are other fluoroplastics other than perfluorinated. Polyvinylidene fluoride (PVDF, structural unit: –CF2CH2–), is an analog of PTFE with half the fluorines. PVF ( polyvinyl fluoride, structural unit: –CH2CHF–) contains one one-fourth the fluorines of PTFE. Despite this, it still has many properties of more fluorinated compounds. PCTFE ( polychlorotrifluoroethylene, structural unit: –CF2CFCl–) is another important compound. It differs from PTFE by having a quarter of fluorine replaced with chlorines, yet this difference brings even greater hardness, creep resistance, and moisture persistence.
Mild fluorination of polyethylene gives does not make all of the plastic lose its hydrogens for fluorine; only a thin layer (0.01 mm at maximum) is then affected. This is somewhat similar to metal passivation: the bulk properties are not affected, but the surface properties are, most notably, a greater vapor barrier. Therefore, they are a cheaper alternative to the perfluoro plastics if only surface is important.
Nafion is a structurally complicated polymer. It has a PTFE-like backbone, but also contains side chains of perfluoro ether that end in sulfonic acid (–SO2OH) groups. It also possesses great chemical stability, while exact properties vary with morphology. However, because of the difficult chemical structure, it is also relatively easily converted to an ionomer (shows conductivity) by adding cations like Na+ or by converting into the sulfonic acid rather than the given sulfonyl fluoride. The conductivity is due to that the main carbon chain separates from the side chains, thus forming polar and non-polar regions. This form is also very hydroscopic.
Fluoroelastomers, like other elastomers (artificial rubbers), consist of disordered polymer chains connected in three dimensions. The main challenges in making fluorelastomers are cross-linking (reacting the unreactive polymers), as well as removing the HF formed during curing. There are three main families of fluoroelasters. VDF/HFP is a copolymer system of vinylidene fluoride and (at least 20%) hexafluoropropylene. TFE/propylene is another copylymer system with better chemical resistance to some solvents. TFE/PMVE (perfluoromethylvinyl ether) is a copolymer system which creates a perfluorinated fluoroelastomer.